CRISPR stands for Clustered Regularly Interspaced Short Palindromic Repeats, which is a revolutionary genetic technology that allows scientists to make precise edits to DNA. It is based on a naturally occurring defense mechanism found in certain bacteria that allows them to recognize and destroy invading viruses by cutting their DNA.
The CRISPR-Cas system works by using an RNA molecule to guide an enzyme called Cas9 to a specific location in the DNA, where it cuts the DNA strands. This cut can then be used to either delete, replace, or insert new genetic material at the desired location. This technology has the potential to transform a wide range of fields, including medicine, agriculture, and biotechnology.
CRISPR has already been used to treat genetic diseases such as sickle cell anemia and cystic fibrosis in preclinical studies, and has the potential to revolutionize the field of gene therapy. In agriculture, CRISPR can be used to create crops that are more resistant to pests and diseases, and that have improved nutritional content. The technology also has potential applications in environmental conservation and biofuel production.
Despite its potential benefits, the use of CRISPR also raises ethical and safety concerns, such as the possibility of unintended genetic changes or the creation of new and potentially harmful organisms. However, with careful regulation and responsible use, CRISPR has the potential to transform the way we approach some of the most pressing challenges facing society today.

The history of CRISPR technology is a story of scientific discovery that spans several decades and involves the contributions of many researchers. Here are some of the key milestones in the development of CRISPR technology:
- 1987: The discovery of CRISPR: In 1987, a group of researchers studying the genome of the bacterium E. coli noticed a series of repeating DNA sequences separated by unique “spacer” sequences. These sequences were later named clustered regularly interspaced short palindromic repeats, or CRISPR.
- 1993: The first CRISPR-associated gene is identified: In 1993, researchers identified the first CRISPR-associated gene, cas1, which was found to be conserved across many different bacteria.
- 2005: The role of CRISPR in bacterial immunity is discovered: In 2005, researchers showed that CRISPR plays a crucial role in bacterial immunity by protecting against viral infections. They found that bacteria incorporate short pieces of viral DNA into their CRISPR arrays, which allows them to recognize and destroy the viral DNA if it enters the cell again.
- 2011: CRISPR is repurposed for genome editing: In 2011, researchers showed that the CRISPR system could be repurposed for genome editing by using a modified version of the Cas9 protein to cut DNA at specific sites in the genome. This allowed for precise and targeted editing of genes in a variety of organisms, including human cells.
- 2012: CRISPR-Cas9 is demonstrated in human cells: In 2012, a team of researchers showed that the CRISPR-Cas9 system could be used to edit the genome of human cells. This breakthrough opened up new possibilities for using CRISPR in biomedical research and gene therapy.
- 2013: CRISPR is used to correct genetic mutations: In 2013, researchers used CRISPR to correct a genetic mutation in human cells that causes a rare genetic disorder called Fanconi anemia. This marked the first time that CRISPR had been used to correct a genetic mutation in human cells.
- 2015: CRISPR-Cas9 is used in agriculture: In 2015, researchers used CRISPR-Cas9 to edit the genome of crops, including tomatoes and wheat, to improve their yield and resistance to pests and disease.
Since these breakthroughs, CRISPR technology has rapidly advanced, with new applications and improvements being developed every year. It is now widely recognized as one of the most promising and powerful tools in modern biotechnology.
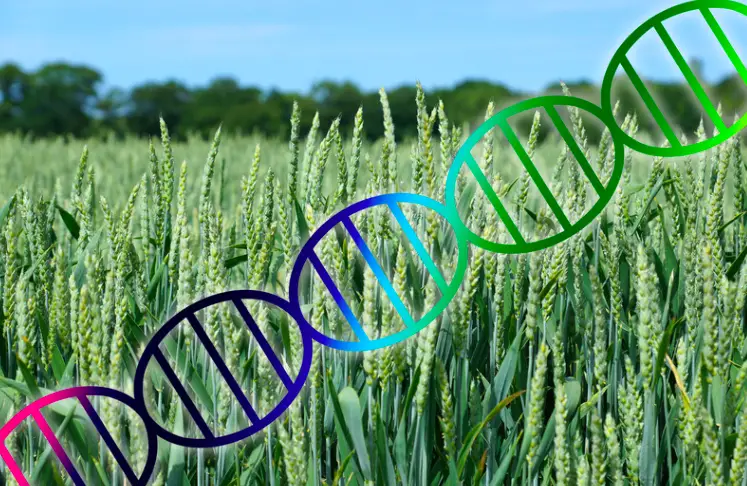
CRISPR is a new genetic technology that differs from traditional genetic engineering in several key ways.
Firstly, traditional genetic engineering relies on a process called transgenesis, which involves inserting foreign genetic material into an organism’s DNA. This foreign genetic material is typically derived from a different species, and the insertion is often random and imprecise. In contrast, CRISPR allows for precise editing of an organism’s own DNA, without the need for foreign genetic material.
Secondly, traditional genetic engineering often relies on trial and error, with scientists selecting the most desirable traits after many rounds of experimentation. CRISPR, on the other hand, allows for targeted and precise editing of specific genes, allowing scientists to achieve the desired trait with greater accuracy and efficiency.
Thirdly, traditional genetic engineering often involves the use of viral vectors or other potentially harmful methods to deliver the foreign genetic material into an organism’s DNA. CRISPR, on the other hand, uses a naturally occurring bacterial defence mechanism that is much safer and more precise.
Finally, traditional genetic engineering has been the subject of much controversy due to concerns about safety and potential unintended consequences. CRISPR, while still relatively new and untested, has the potential to avoid many of these concerns by allowing for precise and targeted editing of specific genes.
In summary, CRISPR differs from traditional genetic engineering in that it allows for precise editing of an organism’s own DNA, without the need for foreign genetic material, and uses a safer and more precise delivery mechanism. While both technologies have potential benefits and risks, CRISPR represents a major step forward in the field of genetic engineering.

CRISPR technology really has the potential to revolutionize the field of food microbiology by allowing scientists to precisely manipulate the genomes of foodborne pathogens, spoilage organisms, and probiotics. Here are some applications of CRISPR in food microbiology:
- Pathogen detection: CRISPR-based diagnostic tests can be developed to quickly and accurately detect foodborne pathogens, such as Salmonella, Listeria, and E. coli, in food samples. This can help prevent outbreaks of foodborne illness by allowing for rapid identification and response.
- Pathogen control: CRISPR can be used to modify the genomes of foodborne pathogens to make them less virulent or more susceptible to antibiotics. This could be used to reduce the risk of foodborne illness by decreasing the ability of pathogens to cause disease.
- Spoilage prevention: CRISPR can be used to modify the genomes of spoilage organisms, such as yeast and bacteria, to increase their tolerance to environmental stressors, such as temperature and pH. This could help prevent food spoilage and extend the shelf life of food products.
- Probiotic engineering: CRISPR can be used to modify the genomes of probiotics, such as lactic acid bacteria, to enhance their health benefits. For example, probiotics could be engineered to produce specific bioactive compounds that promote gut health.
- Crop improvement: CRISPR can be used to modify the genomes of crops to enhance their resistance to pests and disease, increase their nutritional value, and improve their yield. This could help address global food security challenges by increasing the productivity and sustainability of agriculture.
While the applications of CRISPR in food microbiology are still in the early stages of development, the technology has the potential to transform the way we approach food safety, quality, and nutrition. By enabling precise and targeted genetic manipulations, CRISPR could help address some of the most pressing challenges facing the food industry today.